Abstract
The Dead Sea, a hyper-saline lake in the rift valley between Jordan and Israel, is an extreme body of water in many ways. In particular, its salinity is extremely high, resulting in unique biogeochemistry and optical properties.In the spring of 2004 we conducted two days of physical and optical measurements in the lake measuring temperature, filtered and unfiltered, spectral absorption and attenuation, near forward scattering and attenuation and scattering at three angles in the back direction. The lake exhibited a two-layer structure with a warm and more turbid layer at the top 20-30 m and a clearer colder layer below. Both the particulate and dissolved absorption are well approximated by exponentially decreasing functions with the spectral slope of the particulate absorption about half that of the dissolved fraction. Both have similar magnitudes in the blue. Particle size increased with depth consistent with halite crystals growing as they settle. A factor of 3 (!) difference in beam attenuation was observed between instruments having acceptance angles of 0.027 and 0.93 degrees, consistent with the observed domination of particles >30 µm. Thus, our measurements suggest that particulate salt crystals play a significant role in the lake’s
optics. Dissolved salts affect the water index of refraction significantly, requiring a novel processing approach to obtain dissolved and total inherent optical properties from the measurements. To further study this issue, we performed a laboratory analysis of a Dead Sea sample using a spectral attenuation and absorption meter and an instrument measuring attenuation and near-forward scattering. Using a spectral decomposition of the spectral absorption and attenuation, and values published by Sullivan et al. (2006), we were able to get an estimate of the water’s salinity (in NaCl equivalent units) and observed an interaction between dissolved salts and the temperature effect on salt-water absorption that, to our knowledge, has not been previously observed.
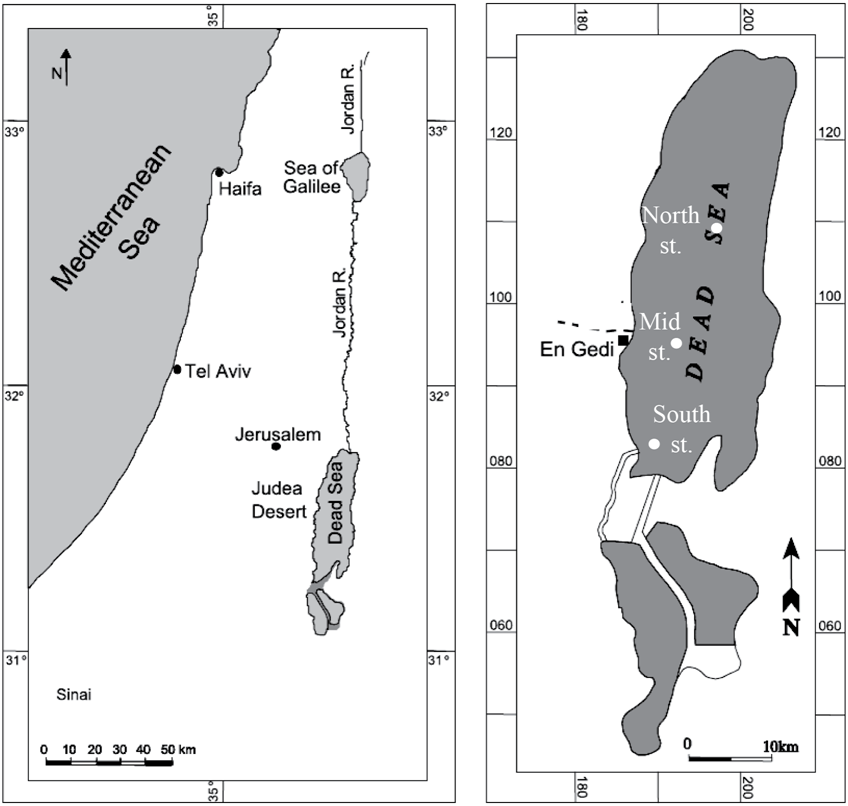
Figure 1. Location of the Dead Sea and the three stations sampled (White dots). Figure slightly modified from that in Kiro et al., 2008.
Introduction
The Dead Sea is a hypersaline terminal lake located in the Jordan Rift Valley (Fig. 1). The lake is 67 km long, up to 18 km wide, and 300 m in depth at its deepest point (Gertman and Hecht, 2000). It is the lowest water body on Earth. Diversion of fresh water upstream the Jordan River since the 1960’s result in a negative water balance, and the lake surface level is dropping at an average rate of 1 m/yr (in 2005 lake level has reached 418 meters beneath sea level, Gavrieli et al., 2006). The lake is extreme in its salinity (approximately 280ppt; Gertman and Hecht, 2002), and density (1.236 kg L-1; Gertman and Hecht, 2002) and, as a consequence of high salinity, almost completely lacks living organisms, except for infrequent and short periods of dense microbial blooms at time of fresh water input (Oren, 1999). Suspended matter in the lake consists mainly of crystals of sodium chloride (halite) that spontaneously precipitates out of the water column (Steinhorn, 1983; Anati, 1993; Gavrieli, 1997; Stiller et al., 1997); In addition, Gavrieli (1997 and references therein) and Stiller et al. (1997) report of observations of suspended crystals of gypsum and aragonite, as well as a small component of detrital sediments (calcite, dolostone, limestone, quartz, feldspars and clay minerals) which are input from dust deposition and rivers. The only optical data regarding the Dead Sea that has been published to date are three PAR irradiance depth profiles (Oren and Shilo, 1982). Given this lack of optical characterization, a short field campaign was organized in the spring of 2004. The measurements presented special challenges, particularly with respect to the use of
deionized water as calibration fluid for the optical instruments used in the lake. Additional laboratory measurements of optical properties of a sample of Dead Sea waters in 2012 allowed us to better understand the effects of salts on the field measurements and on the optical properties of the lake.
Methods
1. Profiling package:
An IOP package containing a WETLabs ac-9, a WETLabs Eco-VSF, a SeaBird CTD (SBE-19+), a pump and a WETLabs DH4 data logger was profiled in the Dead Sea at three different locations (Fig. 1). The Southern station was shallow (~30 m) and near the southern end of the lake overlooking the pipeline connecting the lake with the evaporation ponds of Dead Sea Work at the southern basin of the lake. The two other stations (Middle and Northern) were deep (>250 m depth). The package was profiled with and without a 0.2 µm filter so that the properties of particulate and dissolved materials could be separated. In addition, by differencing the two measurements, we obtained particulate properties that are calibration-independent (Boss et al., 2007, Slade et al., 2010, see below). The package was turned on and lowered to about 10 m for > 5 minutes to warm up the instrument, de-bubble, and acclimate to the local conditions. It was then brought back to the surface and profiled using a winch at a rate of nearly 0.5 m s-1. At the Middle station a LISST-100 (Type B) was also deployed. The LISST had an uncalibrated pressure sensor that was used to determine the depth of the measurements (vicariously calibrated using the fact that the first measurements were within 1 m off the surface while the deepest measurements were at 200 m). The LISST was parked at fixed depths for approximately 1 min so that O(100) measurements could be averaged, decreasing the uncertainty in the mean particulate size spectra. The depth interval was 3 m from surface to 30 m and a 10 m interval below.
2. Data processing steps:
The data from the various sensors were merged in time into a single file using the timestamp provided to each data point by the data logger. Prior to merging, a 3-point median filter was applied to remove spikes from the ac-9 data. CTD pressure was tarred and offset to be co-located with the position where water was pumped into the ac-9 and where the backscattering measurements were done (0.9 m from the top of the cage). Pressure was converted to depth assuming a constant density for the Dead Sea, ρ=1,240
kg m3, 𝑧 = ∙ .=𝑃[𝑑𝑏]/1.216. The associated uncertainty in depth is less than 5% (based on density stratification in the Dead Sea, e.g., Gertman and Hecht, 2002).
Downward profiling data were median-binned into 1 m intervals and calibration offsets were applied based on the median of 6 a-side and 9 c-side calibrations performed within 2 days of the measurements. Uncertainty in the calibrations values, estimated from the standard deviation of different calibrations with deionized water performed within a few days of the deployment ranged spectrally from 0.01−0.02 m-1 for absorption and 0.02−0.03 m-1 for attenuation with largest uncertainties in blue wavelengths. Given the larger uncertainties in the calibration coefficients for the attenuation measurements, CDOM was inferred using the absorption measurements (attenuation measurements were consistently about 0.02 m-1 lower).
a. Particulate properties
Particulate absorption and attenuation were calculated from the difference between 1 m binned total and dissolved measurements resulting in properties that are calibration, temperature, and salinity independent (Boss et al., 2007).The resultant absorption spectrum was featureless but needed to be corrected for scattering. Given that the absorption spectra were expected to be dominated by non-algal
particles (NAP), whose spectra are nearly decreasing exponentials (e.g. Babin et al., 2003), we did not want to assume a lack of absorption at 715 nm, the longest wavelength available with the ac9 (e.g. Zaneveld et al., 1994). Instead, we fit the absorption spectra to the following implicit model: a λ =a 400exp-sλ-440 +βc λ-a λ , obtaining 𝑎 400 , 𝑠, and 𝛽 via a nonlinear least-squares minimization. We then compute the corrected spectra as: a , λ = a λ-βc λ-a , λ . We found that 𝛽~0.16 − 0.23 and 𝑠~0.01 nm-1, consistent with previous estimates and observations for the values of these parameters (e.g. Zaneveld et al., 1994; Babin et al., 2003).
b. Dissolved properties
Dissolved properties were computed from measurements with 0.2 µm filters positioned at the intakes of the ac-9. However, given the higher confidence in the a-side calibrations, we used only the 0.2 µm measurements through the a-side of the ac-9 to estimate CDOM.Values at 650, 676 and 715 were replaced by fitting a λ at shorter wavelength using an exponential fit (from which the spectral slope of CDOM, sg, was computed), and extrapolating to the longer wavelengths. This avoids the problems associated with temperature and salinity effects on water absorption at those wavelengths.
c. Particulate backscattering (Eco-VSF)
We computed the backscattering coefficient at 660 nm using the Eco-VSF following the protocol as in Boss et al. (2004), using Zhang and Hu (2009), to compute the backscattering by dissolved salts assuming S=270 ppt (see Appendix B), integrating over the three angular measurements. Boss et al. (2004), based on closure between different instruments measuring backscattering in different ways at a coastal area, suggested the uncertainty to be on the order of 10%. Effects of dissolved salt on the angle of scattering (through refraction of the light beams at the instrument-water interface) are likely to add some additional small uncertainty to this value.
d. Particulate size distribution (LISST)
We added 0.06 m-1 to the attenuation measured by the LISST to account for transmission offset and salt scattering based on the offset we observed in the lab (see Appendix B). We used both spherical (Agrawal and Pottsmith, 2000) and non-spherical (Agrawal et al., 2008) inversions to obtain particulate size distribution. Where possible, we provide repeat measurements or the same measurements but with different calibration values, to showcase the uncertainty in the values we obtained.
Results and discussion
The Dead Sea exhibited a 1.5 °C strong thermocline separating the surface layer from the isothermal layer below (Fig. 2-4, upper-left panels). Having no salinity measurements, we do not know the exact pycnocline strength. Based on measurements from previous years (e.g., Gertman and Hecht, 2002), temperature and density stratification have a similar pattern. Below 40 m, optical properties are nearly constant while varying continuously in the near-surface layer, suggesting variance in optical properties is driven by near-surface processes.
Particulate properties:
Particulate absorption resembled that of non-algal particles (NAP) having little in way of spectral feature and decreasing exponentially from blue to red (Fig. 2-4). Spectral slopes of particulate absorption were found to be sd~0.01 nm-1 consistent with oceanic NAP. Particulate beam attenuation is featureless as well, being well fitted by a power-law function.Particulate attenuation and absorption were significantly higher at the shallow southern station than at the other two stations. They are also higher in the surface layer compared to at depth with a sharp narrow peak value at the thermocline (~20 m). Surface values of the spectral slope of particulate attenuation (γ) were similar at all stations and relatively high, consistent with smaller average particles near the surface than at depth. The particulate backscattering ratio was reduced with depth suggestive of less refractive particles with depth though the magnitude of difference can be explained simply from the change of size (See Fig. 1a in Twardowski et al., 2001). The beam attenuation of the LISST, measured only at the Middle station, had a similar trend with depth as that of the ac-9 (Fig. 5). It is, however, significantly higher (factor of 3!), which is consistent with its acceptance angle being much smaller (Boss et al., 2009), and of magnitude previously observed (Nukermans et al., 2012). For this to be the case, we would expect the presence of large particles (>30 µm) which do not affect the ac-9 measurements as much as the LISST (Boss et al., 2009). Indeed, the LISST particle size distributions (Fig. 6) exhibited the presence of a population of large particles at all depths, and the relative contribution of this population increases with depth. The increase of size with depth is also consistent with the trend observed with the spectral slope of particulate attenuation (γ). Large beam attenuation values at the base of the thermocline could be the result of the accumulation of large particles on a density discontinuity. However, the outlier Particles Size Distribution (PSD) inverted there (Fig. 6) suggests that it may have been an artifact due the strong change in water index of refraction there which causes the instruments beam to fluctuate away from the detector (Mikkelsen et al., 2008).
Dissolved properties:
Dissolved absorption values were very consistent throughout the Dead Sea, having amplitude that varied by less than 20% from surface to 300 m depth, being higher at the surface layer (Fig. 2-4). Spectral slope was relatively high (0.019±0.001 nm-1), increasing with depth. The lower spectral slope and higher absorption values near the surface were consistent with the surface CDOM having higher molecular weight, being relatively fresher compared to depth (Yacobi et al., 2003).
Comparison with historical data:
The only published optical data we are aware of were collected using a PAR radiometer (Fig. 4 in Oren and Shilo, 1982) spanning April to October of 1980 and collected near the Middle station. The diffuse attenuation of PAR based on fitting a line to these measurements is 0.1±0.01 m-1, which is consistent with the absorption and backscattering measurements we obtained in the current study (a(490)+bb(490)=0.09±0.015 m-1, Figs. 3 and 4).
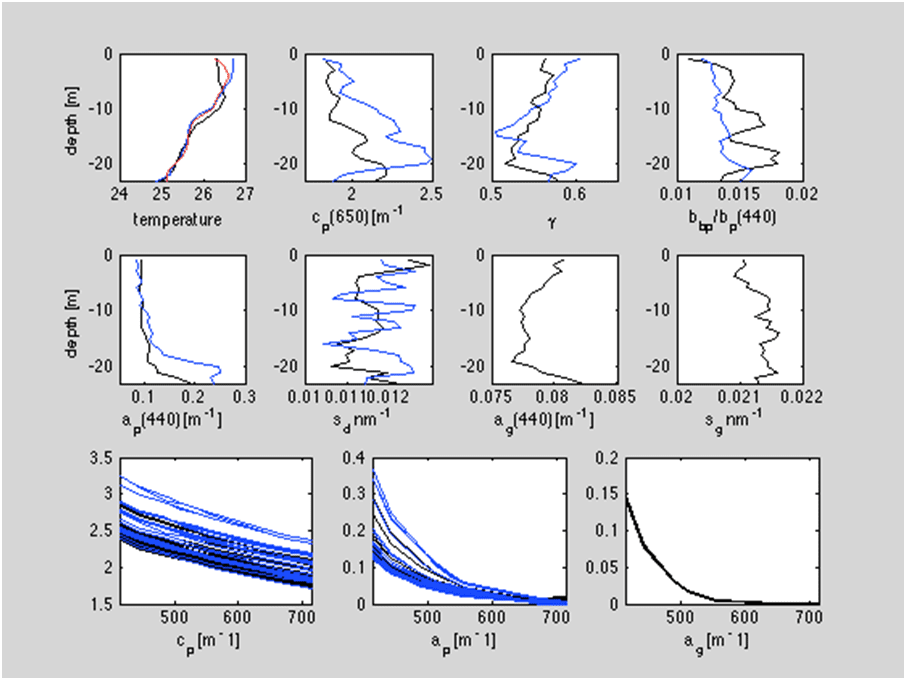
Figure 2. Temperature and Particulate and dissolved IOPs in the Southern station near the connection to the evaporation ponds. From right to left and top to bottom, the properties displayed are: temperature, beam attenuation at 650 nm, the spectral slope of beam attenuation, backscattering ratio, particulate absorption at 440 nm, spectral slope of particulate absorption, dissolved absorption at 440 nm, the spectral slope of dissolved absorption, particulate beam attenuation spectra, particulate absorption spectra, and dissolved absorption spectra. We have two estimates of particulate properties (blue and black lines) based on the difference between two casts performed within 12 minutes of each other where total measurements were taken, and one cast performed in between them, where the dissolved properties were measured.
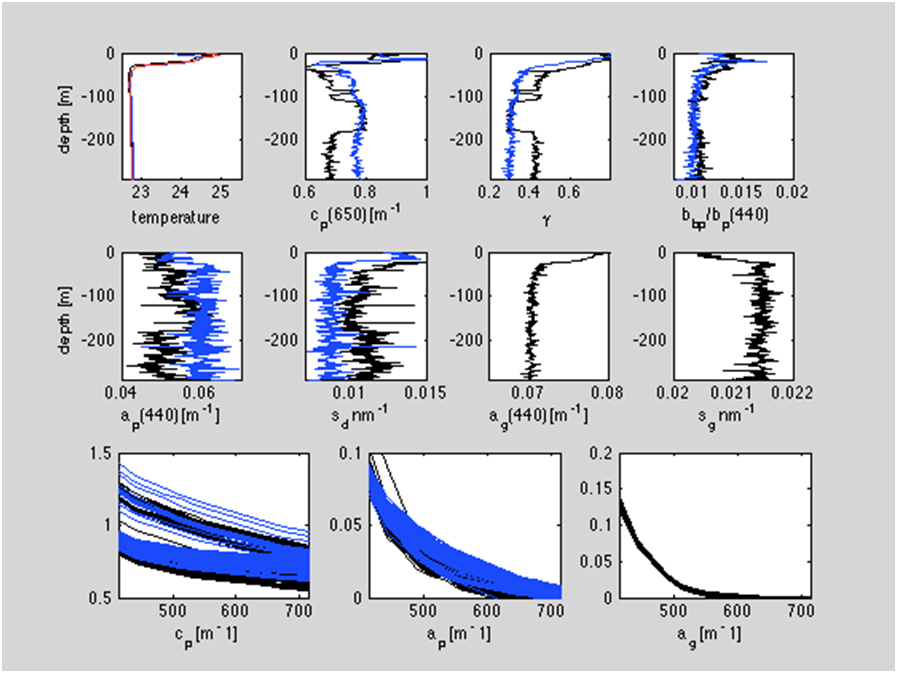
Figure 3. Same as in Fig. 2 but for the Northern station. We have two estimates of particulate properties based on the difference between two casts performed within 45 minutes of each other where total measurements were taken, and one cast performed in between them, where the dissolved properties were measured. Note that while both profiles reveal similar range of properties, both exhibit significantly different distributions of beam attenuation. Large discontinuities in the black profile are inconsistent with other properties and suggest an artifact.
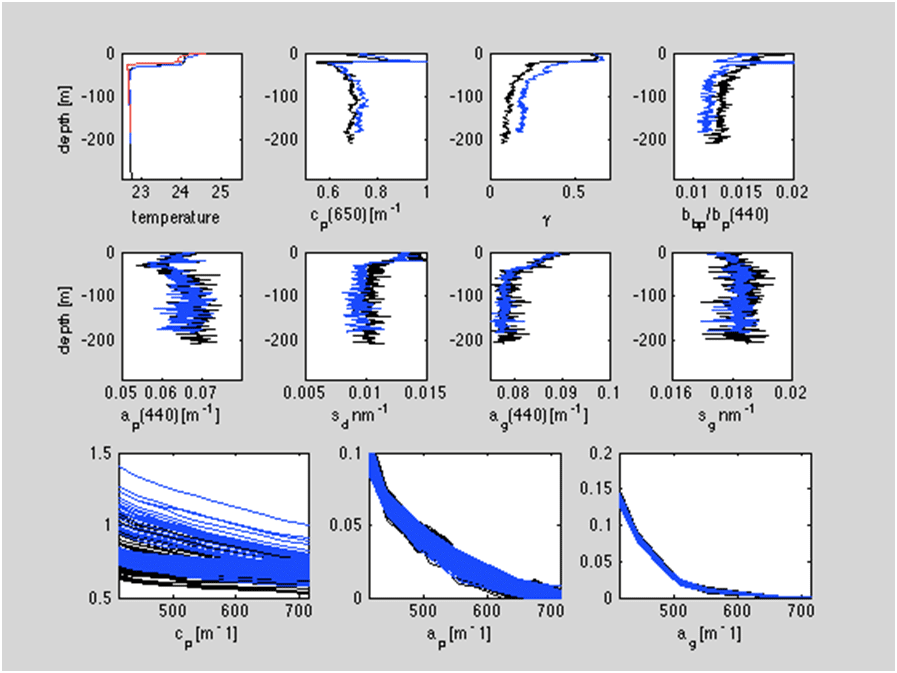
Figure 4. Same as Fig. 2 but for the Middle station. We have two estimates of particulate properties based on the difference between two casts performed within 3 hours of each other where total measurements were taken, and two casts performed in between them, where the dissolved properties were measured. Differences between values here and in Fig. 3 provide an estimate of uncertainty.
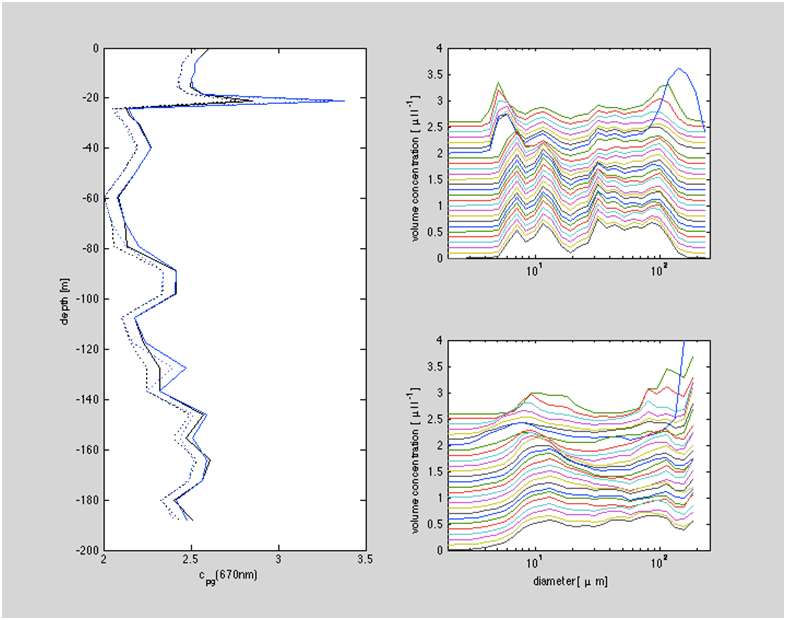
Figure 5. Left panel: LISST Beam attenuation at 670 nm. Solid lines denote the average while the dashed line denotes medians processed with a pre- (black line) and postv(blue) calibration. Right panels: Volume concentration as inverted from LISST measurements using the standard (spherical) inversion (top) and non-spherical inversion (bottom). The curve represents PSDs from different depths with top being near surface and the bottom ~190 m depth. Each curve is 0.1µL L-1 offset from the next, and was taken at an interval of approximately 3 m in the top 30 m and 10 m below. The outlier comes from the thermocline (~20 m) where a strong Schlieren effect may have been present (Mikkelsen et al., 2008).
Summary
This paper presents the first observations of IOPs conducted in the Dead Sea (and possibly in a hypersaline lake). Absorption spectra of dissolved and particulate material are consistent with those observed in the oceans and in fresh lakes, with, however, one major difference, the lack of observable phytoplankton pigment absorption near the surface. Attenuation spectra are also consistent with observations from another aquatic environment, exhibiting power-law-like spectra with no spectral features. A large decrease in spectral slope of particulate attenuation is observed with depth with relatively little change in attenuation magnitude. The likely cause is the formation of larger particles with depth, most likely due to halite precipitation (Anati, 1993; Gavrielli, 1997; Stiller et al., 1997), which scavenges some of the smaller particles.
Acknowledgments
We thank Eli Biton, Alon Shepon, Tal Berman, and the skipper of the “Sivan” for their help during the cruise. Much of the equipment and Boss’s work on particulate scattering were funded by the Office of Naval Research.
Appendix A: Theory: effect of dissolved salts on spectrophotometric measurements
Dissolved salts modify the inherent optical properties of the waters in which they are dissolved (e.g., Morel, 1974; Zhang and Hu, 2009; Sullivan et al., 2006). In addition, they modify the transmission properties at interfaces (e.g., Bohren and Huffman, 1983). We use theoretical considerations to evaluate how IOPs of hypersaline water can be retrieved from instrumentation for which DIW was used as a blank.
When light goes through a collimated design beam-transmissometer it interact with two fluid-window boundaries. At these boundaries some of the light is reflected backwards. The transmission through a boundary between two media 1 and 2, (𝑇=1 −𝑅 , where 𝑅 is reflection), depends on the index of refraction of the two media (e.g., Bohren and Huffman,
Appendix B: Laboratory measurements of a sample of Dead Sea water
A surface sample from the Dead Sea collected on 5/1/2012 near the Southern station (Fig.1) was sent to the University of Maine. The sample was filtered through a 0.2 µm filter. The sample density was measured to be 1,242 kg/m3 consistent with previous accounts of Dead Sea density (e.g. Steinhorn, 1983; Gertman and Hecht, 2002).
a. Measurements with LISST:
During two separate days we performed measurements with Sequoia Scientific’s LISST 100-X of both laboratory DIW and a filtered sample from the Dead Sea. We found that:�� .,= -0.06±0.03 m-1. The large uncertainty is due to the short path length of the sensor as well as inhomogeneities in the Dead Sea waters which we have found to spontaneously precipitate visible crystals after filtration over time scales of hours. The presence of an attenuation offset is due to both scattering by salts (a positive offset) and transmission effects (a negative offset). Absorption due to dissolved material in these waters is negligible at the measurement 670 nm, the LISST wavelength (see below, measurement with ac-s). We use the Quan and Fry (1995) formulation of index of refraction of salt water and Zhang and Hu (2009) to compute the likely salinity that will results in such values:and find that the values of the offset are consistent with S = 200±100 g kg-1. Note that the transmission term dominates, contributing more than 97% to the total attenuation at all salinities. The specific composition of the dissolved salts makes a difference both for the
optical properties of the mixture (e.g., index of refraction and scattering, Zhang et al., 2009) as well as for its physical properties (e.g., density, Anati, 1999). Differences between NaCl and Dead Sea brines are on the order of 10% for water-salt mixture density determination (Zhang et al., 2009). In addition, scattering by salts is not a linear function of their concentration but rather decrease with increasing concentration (Zhang and Hu, 2009; Zhang et al., 2009). We added polystyrene beads of different sizes to dissolved Dead Sea waters to see how much effect salt will have on the LISST inversion. Two effects were expected: 1. Change of index of refraction of the medium in a direction that reduces the particle contrast with the medium making particles in salt behave like smaller particles in fresh water; and 2. Smaller refraction of rays at the window in salt water will cause rays arriving at a large angle to the zenith to register in rings that are further from the center, also suggesting smaller particles). Surprisingly, we observe little effect of Dead Sea waters on the inversion, except for one size bead (15 µm), for which we find the inversion of Dead Sea water suspension to suggest the beads are larger than in DIW (Fig B1). In any case, the maximal shift we observe is of one size bin, suggesting we can still use the LISST in the Dead Sea using its
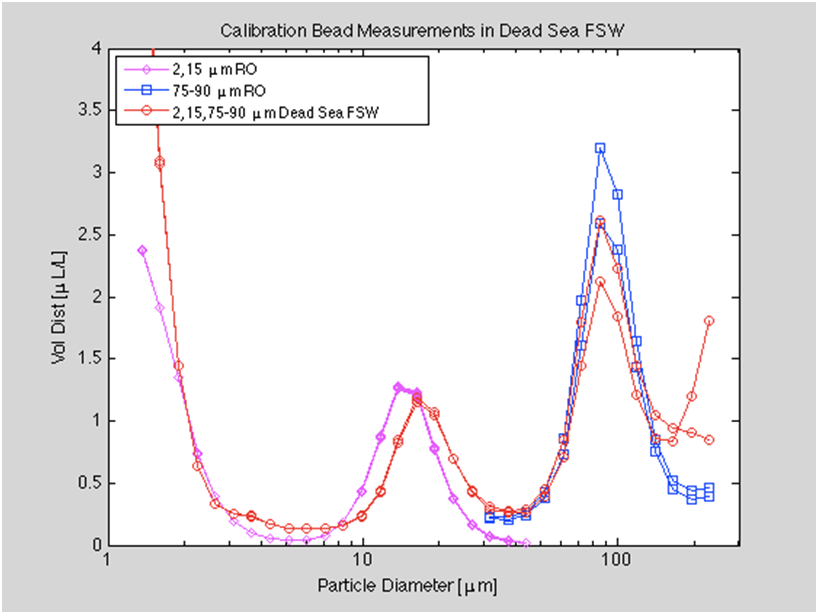
Figure B1. Result of spherical inversion of the LISST for suspensions with beads centered at 2,15 and 83 µm. Note that the most difference observed is associated with one size bin.
b. Measurements with ac-s:
Sullivan et al. (2006) conducted a measurement of the effect of salts (NaCl) on attenuation and absorption of water using a WET Labs ac-s and tabulated their results as a function of wavelength. While Sullivan et al. (2006) accounted for scattering by dissolved salts, they did not explicitly account for changes in transmission. Since the transmission term (3rd term in (A6)) is highly linear as a function of salinity, it should be well captured in those tables. Using their tables, we can write expressions for measured attenuation and absorption by the ac-S as a function of the concentration of salts (S, [g kg-1]): where a term is added to correct of temperature difference (Δ𝑇, 𝜓 is taken from Sullivan et al., 2006), a term for specific scattering by salts (𝑏∗ ) but since scattering within 41.7° are collected by the absorption-side detector of the ac-S the scattering contribution is smaller than to attenuation by a factor 0.166. While temperature has the strongest effect on attenuation and absorption in the NIR, salinity effects are large around 620 nm and scattering by dissolved salts is important in the blue (Fig. B2
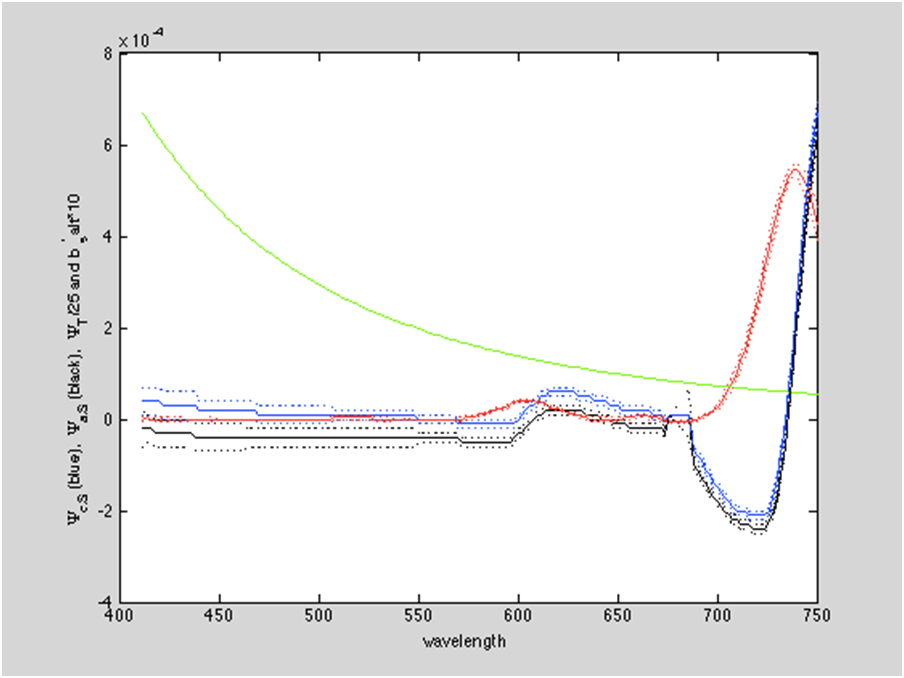
Figure B2. Salt-specific attenuation (blue) [m-1 kg g-1], absorption (black) [m-1 kg g-1], temperature-specific attenuation (red) divided by 25 [m-1 °C-1] and salt-specific scattering (green) multiplied by 10 [m-1 kg g-1]. Dashed lines represent ±1 standard deviation. Scattering data from Zhang and Hu (2009), while all the rest is from Sullivan et al. (2006).
We calibrated the ac-s in the lab using RO water produced by a MiliQ system equipped with a UV lamp and used Sullivan et al. (2006) to correct for temperature difference between lab and manufacturer (as in Slade et al., 2010). Temperature deviation between successive calibrations varied from 0.8 to 3 °C from that of the manufacturer, consistent with a thermometer we used. A surface sample from the Dead Sea was filtered through a 0.2 µm and gently gravity fed through the ac-s. The distinctive salinity signature was observed in the 620-670 nm spectral region as well as the strong effect of temperature (Fig. B3).
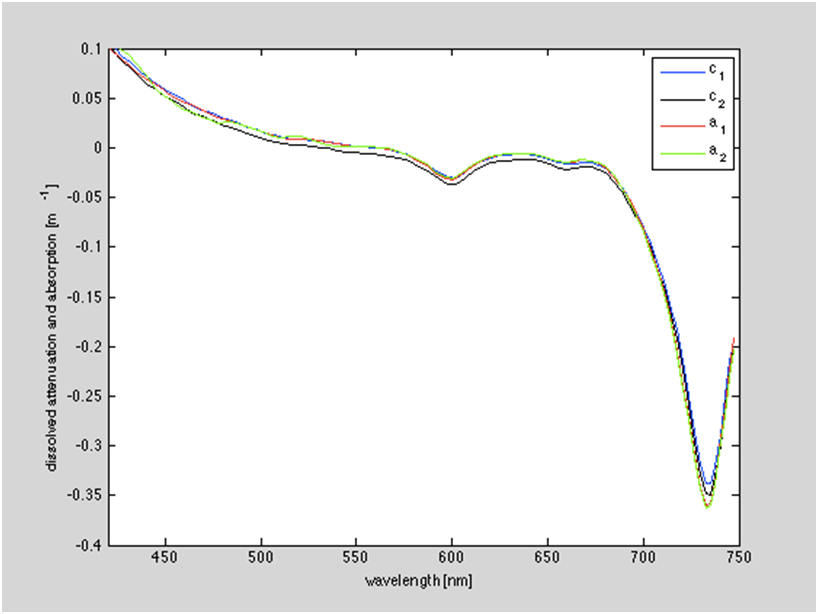
Figure B3. Duplicate measurements of dissolved attenuation and absorption of Dead Sea waters corrected only for DIW offset.
In order to separate these effects, and obtain the absorption and attenuation due to CDOM we used the following model:
The salinity value and density values we obtained (270 g kg-1,1,242 kg m-3) were very close to the values reported in Steinhorn (1983) (276 g kg-1, 1,233 kg m-3), suggesting this absorption decomposition could provide useful salinity information, despite the fact that Sullivan et al.’s (2006) table was based on a solution of NaCl. The high-temperature offset observed (~-28 °C) compared to the calibration fluid
suggests dissolved salts affect how temperature affects water absorption, a process that has not been recognized to date. Finally, we obtained CDOM (𝑐 and 𝑎 ) which are similar in magnitude to those we observed in 2004 (compare Fig. B4 and Fig. 2).
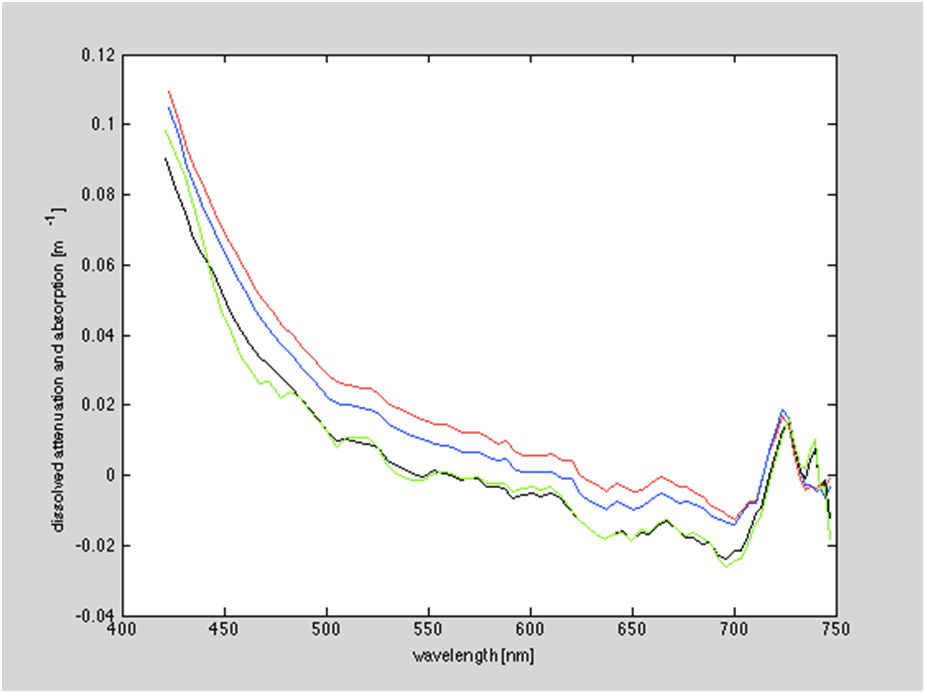
Figure B4. Dissolved absorption (black and green lines) and attenuation (blue
and red lines).